Abstract
In this work the synthesis of thermo-sensitive polymer coated magnetic nanoparticles and their inductive heating have been studied. Poly (N-isopropylacrylamide-co-acrylic acid) (NA) polymers were first synthesized by emulsion polymerization of poly(N-isopropylacrylamide) (NIP) in water and followed by encapsulating magnetic nanoparticles (MNPs). As increasing the concentration of acrylic acid (AA), the lower critical solution temperature (LCST) increased, so that with 150% of AA (molar ratio) the LCST reached 42 °C, which is close to the temperature of hyperthermia treatment. Magnetization and ac susceptibility measurements were conducted to depict some characteristics of the NIP-MNPs and NA-MNPs that are related with the loss power. Attempts to analyze the rate of magnetic inductive heating were performed to show the Brownian relaxation origin of additional heat source created by the magnetite nanoparticles capped with thermosensitive polymers. Our results suggest that these thermo-sensitive polymer-coated magnetic nanoparticles show a potential for hyperthermia and drug delivery application.
Export citation and abstract BibTeX RIS

Original content from this work may be used under the terms of the Creative Commons Attribution 3.0 licence. Any further distribution of this work must maintain attribution to the author(s) and the title of the work, journal citation and DOI.
1. Introduction
During the recent two decades, the fabrication of polymer coated magnetic nanoparticles for various biomedical applications, such as separation and purification [1, 2], drug delivery system [3, 4], magnetic resonance imaging agent [5] and hyperthermia [6, 7] has been performed in many works. A great variety of polymers with functional groups including hydroxyl, carboxyl, amino, etc have been used for these purposes. Among them, thermo-sensitive polymers have been widely studied for drug delivery thanks to their structure change ability at lower critical solution temperature (LCST) [8–11]. At this temperature (32 °C in water solution for poly (N-isopropylacrylamide) (NIP) polymer), the polymeric system undergoes a phase transition so that its volume changes due to the loss of hydrogen bonding [10]. However, the LCST of the polymer should be modified to close to physiological (37 °C) or hyperthermia temperature (42–49 °C) for applying in drug delivery systems and/or cancer treatment [11–13]. The synergistic therapeutic effects of chemotherapy and hyperthermia can be reached thanks to this remarkable property of thermo-responsive polymer [13]. The drug-loaded magnetic nanoparticles with a magnetic core and a thermo-sensitive polymer shell can act as multifunctional agent for combined drug delivery, controlled release and hyperthermia. The loaded drug can be targeted to the tumor using a suitable external magnetic field [14]. Once targeting to the tumor region, an alternating magnetic field (AMF) can be switched on to generate a heat in the targeted nanoparticles to make the local temperature overcome the LCST and that of destroying cancer cells (42–49 °C) [14].
The heat production by the magnetic nanoparticles may be theoretically caused by several mechanisms, among which the two main mechanisms are Néel and Brown relaxations. In Néel relaxation, the energy is dissipated when the moment of the core particle relaxes to its equilibrium orientation. The energy in Brownian relaxation, which is related to the rotational Brownian motion of the encapsulated particle as a whole, is determined by the rotational friction with the suspension fluid. The Brownian, Néel and effective relaxation times (τB, τN and τeff) are given by



where Vh is the volume of the capped particles, η is the viscosity of the surrounding fluids, τ0 is characteristic times, typically assumed to have the approximate value 10−9 s, K is the magnetocrystalline anisotropy constant of the magnetic nanoparticles, Vc is the volume of the magnetic core, k is Boltzmann's constant (1.38 × 10−23 J K−1), and T is the temperature of the system.
The magnetization of the magnetite nanoparticles in a fluid when applied to an AMF can be expressed through the complex susceptibility χ as seen in equation (2). The in-phase component χ' of the susceptibility is proportional to the magnetization while the latter part is directly proportional to a power dissipation in the material
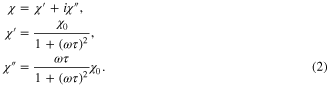
In the case of superparamagnetic nanoparticles in which the hysteresis loss is almost zero, the power dissipation (P) of a ferrofluid in the presence of an AMF is expressed as [15]

where μo is the permeability of vacuum (4π × 10−7 H m−1), χ0 is the initial susceptibility of the system, H0 is the ac field amplitude, and f is the field frequency.
In this report we present some results of fabrication of a magnetic polymeric system that is composed of magnetite nanoparticles (MNPs) in the core and poly (N-isopropylacrylamide) (NIP) polymer in the shell. A transition through LCST can create a change of volume Vh, which, as deduced from equations (1a), (1c) and (2), makes a variation of relaxation time and dissipation that finally influences the power dissipation P (equation (3)). The magnetic property and inductive heating capacity of polymer coated magnetic nanoparticles will be discussed.
2. Materials and methods
2.1. Modification of poly(N-isopropylacrylamide) (NIP) polymer
All chemicals were purchased from Merck and used without further purification. Distilled water was used throughout all experiments. To increase the LCST, NIP was polymerized with acrylic acid via emulsion polymerization. Ammonium persulfate (APS) and sodium dodecyl sulfate (SDS) were used as initiator and emulsifier, respectively; while N, N'-methylene bisacrylamide (MBA) was used as a crosslinking agent. The ingredients for the synthesis of poly(N-isopropylacrylamide-co-acrylic acid) (NA) copolymer are shown in table 1. The polymerization was carried out at 70 °C for 2 h under inert conditions and stirred at 500 rpm. The product was obtained after centrifugation to remove unreacted monomers. The effect of acrylic acid (AA) contents was also investigated with the mole ratio of AA ranging from 50–250%.
Table 1. Ingredients for the synthesis of NA copolymers.
Sample | NIP (mg) | AA (ml) | MBA (mg) | SDS (mg) | APS (mg) | Distilled water (mg) |
---|---|---|---|---|---|---|
NA50 | 0.356 | 0.5 | 0.18 | 0.048 | 0.38 | 50 |
NA100 | 0.356 | 1.0 | 0.18 | 0.048 | 0.38 | 50 |
NA150 | 0.356 | 1.5 | 0.18 | 0.048 | 0.38 | 50 |
NA200 | 0.356 | 2.0 | 0.18 | 0.048 | 0.38 | 50 |
NA250 | 0.356 | 2.5 | 0.18 | 0.048 | 0.38 | 50 |
2.2. Magnetic polymeric system fabrication
Magnetite nanoparticles (MNPs) were first synthesized through co-precipitation process as reported in our previous study [16]. After washing with distilled water until pH reached 7, the product was either dried at 40 °C in vacuum condition for 24 h for characterization or dispersed in water by sonicating for further encapsulation.
To fabricate magnetic polymeric system, MNPs were dispersed in water with a concentration of 1 mg ml−1. The copolymer was then dropped slowly into the MNPs solution under vigorous stirring. The mixture was then stirred for 48 h at room temperature. The un-encapsulated MNPs were removed by centrifugation at 4000 rpm and the magnetic fluid was obtained and kept at room temperature.
2.3. Samples characterization and magnetic heating measurement
To prepare a sample for characterization, 5 ml of magnetic fluid was dried at 40 °C under vacuum condition for 24 h. The structural and morphological characterizations were performed using powder x-ray diffraction (XRD) and field-emission scanning electron microscopy (Fe-SEM). The coating layer of copolymer was verified using Fourier transform infrared (FTIR) spectra. Magnetic properties of MNPs and polymeric MNPs were characterized using a vibrating sample magnetometer (VSM). The LCST was determined by thermo-gravimetric analysis (TGA). The ac susceptibility versus frequency and temperature were investigated by a quantum design physical properties measurement system (PPMS) at 11 Hz and 10 Oe.
Inductive heating capacity of the magnetic polymeric systems was investigated by a system using a commercial generator (RDO HFI 5 kW) at a frequency of 236 kHz and amplitude of 65 Oe. The fluid samples for the magnetic heating measurements were prepared by soluting the polymer capped systems in distilled water at concentration of 5 mg ml−1.
3. Results and discussion
3.1. Structural and morphological characterization
Figure 1 shows the x-ray diffraction patterns of the synthesized MNPs with and without NIP or NA encapsulation. All observed peaks in XRD pattern of the naked MNPs could be attributed to the characteristic diffraction peaks of magnetite [15]. These peaks were also observed in either NIP or NA encapsulated MNPs thus indicating that the encapsulation was not affecting the nature of the magnetite nanoparticles. The average size of MNPs calculated by Sherrer equation [17] was about 12 nm, which is in close agreement with the result obtained from Fe-SEM images (not shown). FTIR spectra of MNPs, NIP-MNPs and NA-MNPs sample are shown in figure 2. The peak at 578 cm−1 is the characteristic peak of Fe–O–Fe in bulk Fe3O4 [17]. This peak was also observed in IR spectra of the NIP-MNPs and NA-MNPs with a slight shift. A broad band in the range 3000–3600 cm−1 was assigned to the stretching vibration of hydrogen bond (O–H....H). Typical peaks for the amide (II) mode present in the NIP and NA-MNPs were observed at 1550 cm−1 while the bands at 1631 and 1621 cm−1 were attributed to stretching vibration of carboxylate group. These results indicate the formation of layer on the surface of magnetite nanoparticles.
Figure 1. XRD pattern of naked MNPs, NIP and NA150 encapsulated MNPs.
Download figure:
Standard image High-resolution imageFigure 2. IR spectra of un-encapsulated MNPs, NIP-MNPs and NA150-MNPs.
Download figure:
Standard image High-resolution imageMorphology of the NIP-MNPs and NA-MNPs was elucidated by field emission scanning electron microscopy (Fe-SEM) and the images are shown in figure 3. From this figure, it can be seen that the obtained particles were almost spherical. Moreover, the average size of the nanoparticles after encapsulation increased in accord with the content of AA in the thermo-sensitive polymer. As for NIP-MNPs, the average size was about 20 nm which was observed to increase to approximately 30, 50 and 70 nm for NA50-, NA150- and NA250-MNPs, respectively. This might be explained so that a higher content of AA could make the nanosystem swell better in aqueous system, thus leading to a larger size of the capped system [13].
Figure 3. Fe-SEM images of (a) NIP-MNPs, (b) NA50-MNPs, (c) NA150-MNPs and d) NA250-MNPs.
Download figure:
Standard image High-resolution image3.2. Effect of acrylic acid (AA)content on the LCST of NA-MNPs
The LCST of NIP polymer could be modulated via copolymerization with acrylic acid. The LCST of NA-MNPs was investigated using thermo-gravimetric analysis (TGA) as can be seen in figure 4. One can clearly see a peak at 32 °C in DTG curves of NIP-MNPs which is corresponding to the transition temperature of the NIP before modification. In the case of NA150-MNPs, two peaks were evidenced, a low peak at 31 °C and a high peak at 41.85 °C. The appearance of the main peak at elevated temperature proves that acrylic acid was quite well polymerized with NIP, thus leading to an increase in transition temperature LCST. The small DTG peak of NA sample indicates a residue of some NIP in the sample that still needs further study to totally eliminate. The effect of AA content on the LCST of NIP and NA coated magnetic nanoparticles is shown in figure 5. From the figure it can be seen that on increasing the AA content, the LCST of NA-MNPs also increases. This is due to the fact that hydrophilic part of AA could enhance the hydrogen bonding between NA-MNPs and H2O thus results in increasing the LCST of NA-MNPs [13]. This result is well consistent with other reports [13, 18, 19]. With the AA concentration from 150% to 250% the LCST of the obtained system was nearly in the range of hyperthermia temperature (i.e. 42–49 °C), hence promising a potential for being used simultaneously as drug delivery and hyperthermia agent.
Figure 4. TGA and DTG curves of NIP-MNPs and NA150-MNPs. Inset: enlargement of the transition temperature region.
Download figure:
Standard image High-resolution imageFigure 5. LCST of NIP-MNPs and NA-MNPs system.
Download figure:
Standard image High-resolution image3.3. Magnetic characterization
The magnetic field dependence of magnetization of the naked MNPs, NIP-MNPs and NA-MNPs is presented in figure 6. The saturation magnetization (Ms) of uncapped MNPs was about 73 emu g−1. NIP-MNPs and NA-MNPs samples exhibited slight decreases in Ms, i.e. 72 and 69 emu g−1, respectively. This reduction could be explained by the occupation of polymer in the polymeric MNPs, with the amount of 4% and 8% for NIP-MNPs and NA150-MNPs, respectively, as indicated by the thermo-gravimetric analysis.
Figure 6. Magnetization hysteresis of naked MNPs, NIP-MNPs and NA-MNPs.
Download figure:
Standard image High-resolution imageFigure 7 shows the temperature dependence of imaginary components χ'' of NIP-MNPs and NA-MNPs. One can distinguish from the χ'' versus T of NIP-MNPs sample a sudden change appearing in the range of 32.5–37 °C. In more details, this χ'' change occurs via two regions of opposite direction, from T = 32.5 to 35 °C, χ'' decreases with an amount of about 5 × 10−5 emu g−1 and from T = 35 to 37 °C it increases with 10 × 10−5 emu g−1. For the case of NA150-MNPs, i.e. magnetite particle capped with modified thermosensitive polymer, the χ'' changes in such a way that it first decreases by about 9 × 10−5 emu g−1 when T changes from 31 to 35 °C, then increases by about 15 × 10−5 emu g−1 for T = 35 to 41 °C, and finally decreases by 6 × 10−5 emu g−1 for T = 41 to 45 °C. We suppose the two reversion times of the χ'' variation to be related with the two peaks in DTG curve observed for the case of NA150-MNPs sample, so that the temperature where χ'' started to decrease might be corresponding with the LCST value depicted from the DTG peak. It is worth noting that the observation of χ'' jump is in agreement with that earlier reported in [20] for the case of pNIPAM coated magnetic nanoparticles.
Figure 7. Temperature response for components of ac susceptibility of NIP-MNPs (left) and NA150-MNPs (right).
Download figure:
Standard image High-resolution imageAs for the impact of χ'' change to the dissipation, at the transition temperature the hydrodynamic diameter Vh of the nanoparticles decreased leading to a decrease in particles volume and thus in Brownian relaxation time τB which resulted in a change of the imaginary component χ'' of the ac susceptibility. Because Δχ'' change depends not only on τB but on if the product ωτeff is smaller or larger than 1, one needs more studies about the dynamic behavior in order to elucidate in more detail the concerned relationship.
Anyway, the impact of Vh change should be reflected qualitatively on the magnetic heating behavior. The specific absorption rate (SAR) is expressed by equation (4) where C is the specific heat of ferrofluid and sample holder taken together, dT/dt is the initial slope of the time dependent temperature curve, mMNPs is the total MNPs content in the fluid [21]

The magnetic heating capacity of the two ferrofluids, NIP-MNPs and NA150-MNPs, was carried out at the field of frequency f = 1/ω = 236 kHz and amplitude H0 = 65 Oe from ferrofluid samples of concentration of 5 mg ml−1. The three characteristic heating parameters estimated for these samples as well as those of Alg-MNPs nanoparticles (a sample of similar core particle capped by Alginate polymer [22]) are gathered in table 2. When comparing, it can be seen that the saturation heating temperature of NIP-MNPs was higher than that of NA150-MNPs at the same concentration of MNPs. This result is consistent with the Ms behaviour which is explained above. The same trend was also observed for the initial heating rate and specific absorption rate.
Table 2. Magnetic heating parameters.
Sample | Saturation heating temperature (Ts, °C) | Initial heating rate (dT/dt, °C s−1) | Specific absorption rate (SAR, W g−1) |
---|---|---|---|
Alg-MNPs | 90 | 0.11 | 113.4 |
NIP-MNPs | 91.4 | 0.12 | 100.8 |
NA150-MNPs | 85.3 | 0.078 | 65.52 |
Figure 8 shows the temperature elevation and its rate versus real time for the NIP-MNPs, NA150-MNPs and Alg-MNPs. As observed, the temperature of all the samples increased remarkably at first and then rose more slowly to some saturation temperature Ts, which reflects the heating performance of the ferrofluids. If the heat source is constant in time, the system temperature approaches Ts via a heat diffusion process, which results in a smooth decrease of the heating rate, dT/dt, as can be seen for the case of Alg-MNPs sample. It is interesting to note that the curves of the heating rate versus evolution time or temperature observed in the cases of NIP- and NA150-MNPs exhibited a clear deviation from the normal heat diffusion behavior. Namely, in the case of NIP-MNPs the dT/dt curve deviation occured in the range between 35 and 68 °C, so that in the subrange from 35 to 63 °C the decrease of dT/dt strongly slowed down when affected by the appearance of some additional heating energy; and the second subrange, from 63 to 68 °C, reflecting a return of the system to the normal heating. We suppose the additional heat energy originated from an increase of the Brownian relaxation dissipation caused by the volume change of the capping polymer NIP. The observed delay in switching off of the process could be caused by macroscopic temperature measurement and/or the differentiation approach, which needs further investigation to clarify. For the NA150-MNPs sample, the deviation of heating rate appeared in a broader temperature range, namely from about 35 to 76 °C (figure 8 and table 3). Although the experimental curve was not so distinguishable, one can assume there existed more than one on/off contribution of the additional heating.
Figure 8. In situ temperature elevation (T) and heating rate (dT/dt) of (a) NIP-MNPs, (b) NA150-MNPs and (c) Alg-MNPs at concentration of 5 mg ml−1.
Download figure:
Standard image High-resolution imageTable 3. Characteristics of heating rate curves.
NIP-MNPs | NA150-MNPs | ||||
---|---|---|---|---|---|
t1 (s) | 35 | Additional heating on | t1 (s) | 35 | Additional heating on/off |
T1(°C) | 35 | T1(°C) | 35 | ||
t2 (s) | 270 | Additional heating off | t2 (s) | 35–700 (250) | Additional heating on/off |
T2(°C) | 63 | T2(°C) | 35–76 (48) | ||
t3 (s) | 350 | Back to normal heating | t3 (s) | 700 | Back to normal heating |
T3(°C) | 68 | T3(°C) | 76 |
Finally, it is worth noting that the SAR of the NIP-MNPs system was about 100 W g−1, which is 35% higher than that of NA150-MNPs ferrofluid (table 2). This difference can be attributed to the weaker hydrogen bonding of NIP-MNPs leading to agglomeration of the nanoparticles when applied under magnetic field, which increased the size of the clusters in aqueous medium. The observed SAR value of NIP/NA150 coated MNPs seems to be much higher than the SAR reported by Krishnan et al [23] for PMAO-PEG coated nanoparticles under the application of a 4 times stronger magnetic field (376 kHz, 162.5 Oe), namely SAR = 80–90 W g−1.
4. Conclusion
In this work the multifunctional magnetic systems of poly(N-isopropylacrylamide-co-acrylic acid)/Fe3O4 were successfully synthesized. The amount of AA was an important factor affecting the size and the lower critical solution LCST of poly(N-isopropylacrylamide-co-acrylic acid)/Fe3O4 nanoparticles. Increasing the acrylic acid content would increase the LCST of the NA-MNPs. With 150% of AA in mole ratio, the LCST of the obtained nanoparticles was about 42 °C which is very close to hyperthermia temperature. The analyses of differential thermogravity, magnetic susceptibility and temperature elevation by magnetic inductive heating attempted clearly to show that a volume change transition of the thermosensitive capping polymer should impact correspondingly the heating characteristics of the magnetic nanoparticles. The fabricated thermo-sensitive coated MNPs exhibited quite strong magnetic heating effect, thus promising a potential for applications in hyperthermia as well as in drug delivery systems.
Acknowledgment
This work was performed under the financial support of the National Foundation for Science and Technology development of Vietnam Grants No. 103.02-2011.31. The authors would like to acknowledge the IMS Key Laboratory for facilities support.